Characteristics and Technologies for UAS Superiority to Human-Piloted Aircraft in Air-to-Air Combat
- Setondji V. Nahum
- Oct 7, 2020
- 11 min read
Updated: Oct 15, 2020
Course: Application of Unmanned Systems
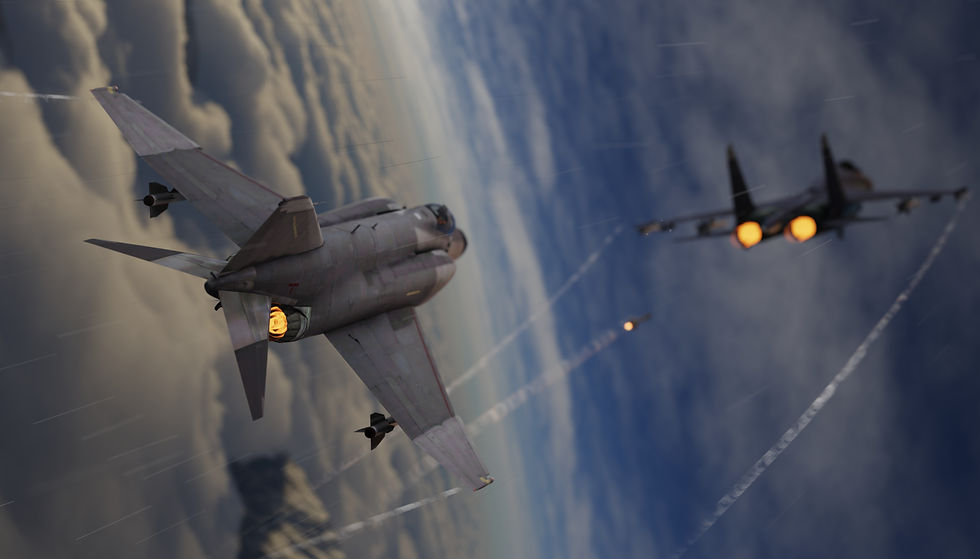
Abstract
Aircraft performance characteristics indicate that unmanned aircraft systems are the result of a design optimization of human-piloted aircraft. Unmanned Aerial Systems (U.A.S.) exhibit improved capabilities and characteristics that render the system more maneuverable (Anderson, 2016). Basic control systems engineering theories indicate that outer loop designs that characterize manned systems in-flight inputs support a slower and often less efficient decision making than autonomous unmanned aircraft systems (Ashokkumar, 2019). The possibility of equipping autonomous aircraft control systems with advanced technologies such as automated approximate dynamic programing systems allows UAS to complete trajectory maneuvers that cannot be completed by humans due to limitations of human physiology. Enemy aircraft maneuvers are predicted and a UAS is kept in position of advantage over adversary’s aircraft (McGrew et. al, 2010).
Air-to-Air combat present significant safety risks including the loss of human lives to manned systems. Fatigue, cognitive loads, physical and social stressors often lead to a loss of situational awareness (Guastello, 2014). A significant percentage of accidents are attributed to crew errors which indicates that human errors in decision-making during air-to-air combat is a significant problem for manned systems and remotely operated aircraft systems (Jaussi et. al., 2018)
Introduction
The man versus machine debate has mesmerized mankind since the dawn of advanced machinery and computerized control systems. The rise of artificial intelligence systems has rendered greater machines capabilities and rapid decision making. This is especially true in the field of aircraft piloting. Aircraft are transportation flying devices which are designed to move people and cargo from one place to another (NASA, 2015). Technological advancements and a focus on continuous improvement in aircraft design has led to the development of unmanned aerial systems (UAS). An UAS is a pilotless aircraft, a flying machine without an onboard human pilot or passengers. Unmanned refers to the total absence of a human who direct and actively pilot the aircraft. Control functions for unmanned aircraft may be either onboard or remote controlled. (Valavanis & Vachtsevanos, 2015, p. 44). UAS comprise individual system elements consisting of an unmanned aircraft, the control station, and any other system elements necessary to enable flight. Such system elements include one or multiple command and control links. It also includes launch and recovery elements (Valavanis & Vachtsevanos, 2015). UAS applications include deep space exploration, search and rescue, and air-to-air combat.
Air-to-Air combat present significant safety risks including the loss of human lives to manned systems. Human physiology restricts manned systems from optimum aircraft maneuvering capabilities. Basic control systems engineering theories indicate that outer loop designs that characterize manned systems inputs support a slower and often less efficient decision making by manned systems in contrast to autonomous unmanned aircraft systems. A significant percentage of accidents are attributed to crew errors which indicates that human errors in decision-making during air-to-air combat is a significant problem for manned systems and remotely operated aircraft systems (Jaussi et. al., 2018).
A testimony to UAS suitability for air-to-air combat is the Kratos XQ-58 A Valkyrie. It is a stealthy high performance unmanned combat aerial vehicle which is built to reach very high speeds with a high maximum range and endurance capabilities. Its physical characteristics include a length of 30 ft., a wingspan of 27 ft. and a height of 8.3 ft. Its cruise speed is reported as 0.72 M with a maximum range of approximately 3000 NM. Kratos reports that its operational altitude is within 50 ft. AGL and 45000 ft. MSL (Kratos, n.d.). It is designed for maneuverability and equipped with a plethora of lethal weapons. Air-to-air Combat UAS shall be equipped with common subsystems such as: power and energy, electrical power distribution, propulsion, vehicle control, maneuver control, trajectory correction, navigation and positioning, obstacle avoidance, vehicle monitoring, health monitoring, communications, payloads, locator, and emergency equipment’s. Supporting technologies shall include radar warning receivers, missile approach warning tool, active electronically scanned array, cognitive radio, electro-optical sensors, infrared cameras, cooled and uncooled infrared detectors (Valavanis & Vachtsevanos, 2015). Air-to-air combat UAS shall be primarily designed to be autonomous but also have the capabilities of being remotely piloted. It is critical that fighter UAS be equipped with a technological tool that computes near optimal fighter maneuvering decisions. What are the most significant characteristics and technologies that shall be developed and implemented by aircraft manufacturer for UAS superiority to human-piloted jet aircraft for air-to air-combat?
Multiple researchers and entities have studied UAS development and usage for air-to-air combat. McGrew et. al report in their research study titled: Air-combat strategy using
approximate dynamic programming that flight maneuver decisions can be planned efficiently by applying approximate dynamic programing (McGrew et. al, 2010). Guastello reports within the textbook titled Human Factors Engineering and Ergonomics that basic conditions such as extreme temperatures, noise, toxins, crowding, isolation, and anxiety are among the main factors contributing to spatial disorientation and illusions in flight (Guastello, 2014). Kelly states within his research study titled Performance Measurement during Simulated Air-to-Air Combat that a pilot who manages speed and altitude well has an advantage over a pilot who does worst at managing such flight characteristics (Kelly, 2016). Trsek highlights in his research article titled The last manned fighter: replacing manned fighters with unmanned combat air vehicles that the Air Force can replace manned fighters with unmanned combat air vehicles and meet mission requirements at lower costs (Trsek, 2008).
Background
Researching the characteristics and technologies that shall be developed and implemented by aircraft manufacturer for UAS superiority to human-piloted jet aircraft for air-to air-combat was completed through documentation analysis. The first step was to research relevant textbooks that discuss flight mechanics, principles of dynamics and control system engineering. The second step was to select a legitimate source of documented research. The third step was to define the criteria for material to be selected. Such criteria were human factors, in-flight maneuvering and decision making, aircraft targeting systems, aircraft control systems design, real-life performance and accident records and program development costs. Per the specified criteria, table 1 displays the textbook and research studies selected.
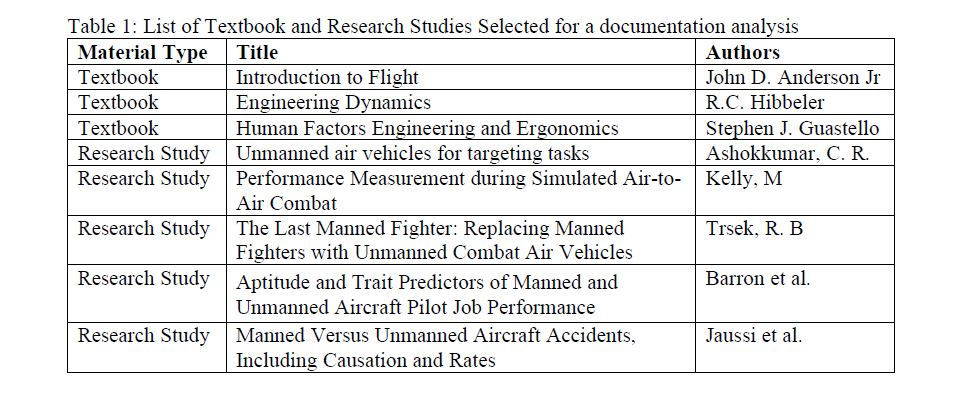
Aerodynamics performance characteristics, aircraft stability and control principles and Newton’s second law of motion are essential to the study of aircraft motion (Anderson, 2016). Figure 1 displays essential elements that characterize aircraft performance. Figure 2 displays Newton’s second law of motion which stipulates that the sum of the forces acting on an element along an axis are equal to the product of its mass and acceleration (Hibbeler, 2007).

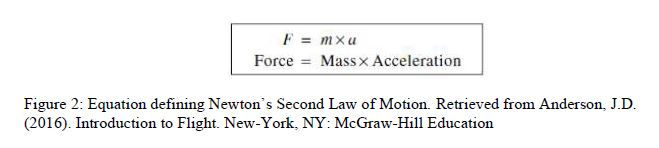
A thorough analysis of aircraft performance shall include specific conditions and elements such as level flight performance, climb performance, range, endurance, take-off, landing and maneuverings. Level flight performance characteristics include the lift coefficient CL and the thrust required TR ( Anderson, 2016).
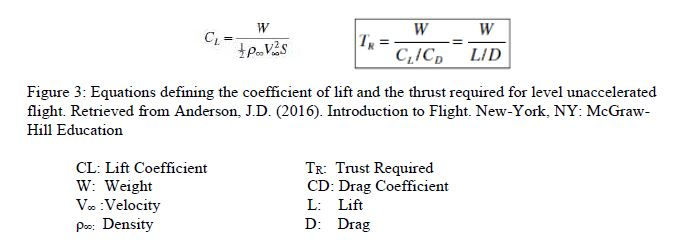
Climb performance is characterized by the rate of climb R/C ( Anderson, 2016)
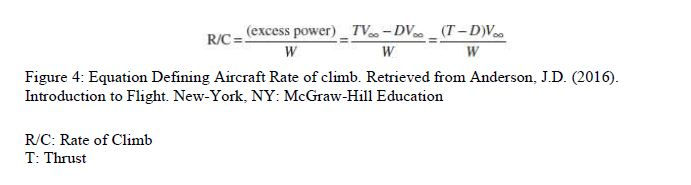
Range and endurance are important characteristic of airplane performance. The Thrust-specific fuel consumption (TSFC) of jet engines depends on the thrust produced by the engine. Maximum endurance is reached when the aircraft is operating at the minimum thrust required. This is the point where the lift to drag ratio (L/D) is at its maximum ( Anderson, 2016).
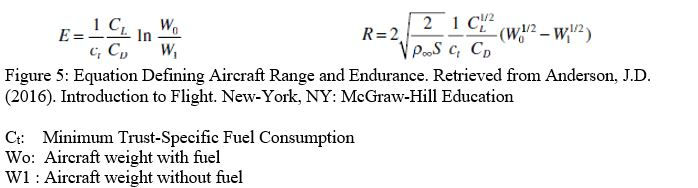
Maneuvering performance of an aircraft is characterized by two important physical quantities: turn radius R and turn rate ω. Aircraft maneuvering performance is optimized with a minimum turn radius R and maximum turn rate ω. This occurs when the aircraft load factor (L/W) is at a maximum with its velocity as low as possible (Anderson, 2016).

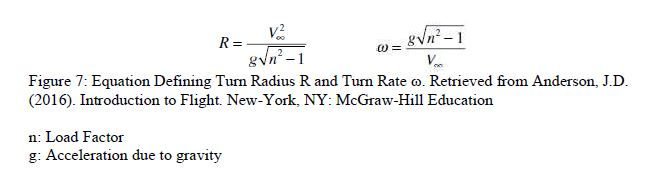
Flight maneuvering decisions, aircraft flight path and trajectories can be optimized for UAS. Basic flight maneuvering can be automated through an approximate dynamic programing method. When applied specifically to air-to-air-combat, this method is capable of predicting adversaries’ maneuvers and provide the required characteristics for a UAS to maintain positions, path angles and trajectories that are advantageous with respect to the enemy aircraft ( McGrew et. al., 2012). Piloted aircraft maneuvering and position inputs are communicated to the aircraft main control systems through an outer loop but autonomous UAS control these flight characteristics through inner loop input. In this case, equilibrium points are switchable with the objective being the completion of autonomous tasks. An analysis based on control system theories indicates that autonomous UAS are at least as effective as manned and remote-piloted aircraft at targeting tasks (Ashokkumar, 2019). Parameters and tests used to evaluate the efficiency and capability of manned systems indicate that unmanned systems are at least as efficient and capable as manned systems. The expected success ratio for unmanned aircraft is predicted to be at least equal to the success ratio of manned systems (Caretta, 2013).
Pilots and crew errors are a significant cause of aircraft accidents and such even in the case of remotely operated aircraft. Interoperability issues between pilots, operators and aircraft control systems have considerably contributed to this issue. The development of fully autonomous unmanned aircraft systems will improve and significantly mitigate aircraft accidents and safety issues (Jaussi et. al., 2018).
Recommendation
Determining characteristics, technologies and factors that render unmanned aircraft systems superior to human-piloted jet aircraft for air-to air-combat requires a mathematical analysis of aircraft performance characteristics and a system approach. Evaluation criteria shall focus on elements that optimize aircraft capabilities. A higher priority shall be given to aircraft performance characteristics, maneuvering performance, aircraft control systems capabilities and technologies, and lower safety risks.
A basic analysis of the mathematical equation in figure 2 which describes Newton’s second law indicates that for a basic system constituting of an applied constant force on a defined element type, the magnitude of acceleration can be tremendously increased by selecting an element with a lower mass. This basic principle can be extrapolated and applied to the evaluation of performance characteristic of unmanned and manned aircraft. In the case of level unaccelerated flight, the lift coefficient and thrust required equation displayed in figure 3 indicates that these quantities can be optimized by lowering the weight of the system. When considering a specific aircraft system, aircraft designers can attain lower systems lift coefficient and thrust required by eliminating the weight of human and human support subsystems (Anderson, 2016).
Equations defining aircraft climb performance, range and endurance are displayed in figure 4 and figure 5. A mathematical analysis of these equations leads to mesmerizing results. The rate of climb, range and endurance of an aircraft system can be significantly optimized by lowering the system weight. When applying this concept to the issue of unmanned versus manned systems, a defined aircraft system’s maximum rate of climb, maximum range and maximum endurance can be significantly increased by eliminating the weight of humans and humans support subsystems (Anderson, 2016). A characteristic that is not automatically improved by lowering an aircraft system weight is the system’ maximum velocity. When considering the characteristic that is wing loading (W/S), lowering the system weight W decreases the wing loading (W/S). This is the parameter that prevent an automatic improvement of the system maximum velocity. This issue can be easily mitigated by lowering the wing area for unmanned aircraft systems (Anderson, 2016). A mathematical analysis indicates that unmanned aircraft system can maintain similar wing loading values to manned aircraft system by simply decreasing the wing area because lowering the denominator of a fraction increases the value of the ratio. With an equal wing loading (W/S) value for a manned and unmanned systems, the lower weight of the unmanned systems directly correlates to significantly higher maximum velocity for the unmanned systems (Anderson, 2016). Therefore, unmanned aircraft systems offer significantly better aircraft performance characteristics than manned systems. Based on the principle and theories of mathematics, unmanned aircraft systems are superior to manned aircraft systems.
Basic control system engineering principles states that inner loop designs generate improved response in term of speed and stability (Nise, 2008). Therefore, autonomous UAS shall be expected to be much quicker at determining an optimum flight path angle and a line of sight engagement than remote piloted aircraft and human piloted aircraft. They shall also be expected to complete the required maneuvering at a higher speed while keeping an optimum path for aircraft stability (Ashokkumar, 2019). This is also supported by a basic mathematical analysis of an aircraft load factor which is defined as lift over weight (L/W). For a defined aircraft system type, the lower weight achievable with unmanned system tremendously increases the value of the ratio. The load factor is a parameter that directly affect aircraft turn radius and turn rate. Figure 7 displays equations defining aircraft turn radius and turn rate. A mathematical analysis of these equations indicated that a higher load factor correlates to a lower turn radius and a higher turn rate. This confirms that unmanned aircraft systems are more maneuverable than manned aircraft. Moreover, the possibility of equipping autonomous aircraft control systems with advanced technologies such as automated approximate dynamic programing systems allows UAS to complete trajectory maneuvers that cannot be completed by humans due to human physiology limitations. This system predicts adversary’s aircraft maneuvers in air-to-air combat and design the ideal counter-maneuvers to keep a UAS in a position of advantage over enemy’s aircraft ( McGrew et. al., 2012). It is then clear that with such systems and capabilities, Unmanned aircraft systems are designed and equipped for superiority over manned systems.

Manned aircraft systems are susceptible to pilots and crew errors. These errors are reportedly the cause of significant accidents and issues in-flight. Despite defined safety standards, human factors such as fatigue, cognitive loads, physical and social stressors often lead to a loss of situational awareness (Guastello, 2014). Basic conditions such as noise, toxins, extreme temperatures, crowding, isolation, and anxiety also contribute to spatial disorientation and illusions in flight (Guastello, 2014). Human factors are a significant safety risk during air-to-air combat. One of the most important safety issues that affect manned systems is the possibility for lost pilot and humans’ lives. In contrast to manned systems, unmanned aircraft systems are not characterized by such system limitations. The lack of a human pilot ensure that fatalities remain on the enemy side. This further indicates that Unmanned aircraft systems shall be preferred over manned systems.
Conclusion
A mathematical analysis of equations defining key aircraft performance characteristics indicate that the elimination of human weight and human support systems weight lead to significant improvement. It optimizes aerodynamics quantities such as lift coefficient, thrust required, rate of climb, range, endurance, turn radius and turn rate. The maximum velocity achievable by unmanned aircraft can be significantly increased by lowering the wing area and thus increasing the wing loading. It is important to note that the lower systems weight of UAS increases the aircraft load factor. This optimizes the UAS turn radius and turn rate and renders unmanned aircraft systems more maneuverable than manned aircraft (Anderson, 2016).
Unmanned aircraft systems are superior to manned aircraft systems. UAS hold a tremendous advantage because they are equipped with technologies that are specifically designed to mitigate human limitations and improve overall system capabilities. They are built with control systems and technologies that allow autonomous unmanned aircraft to predict enemies maneuvers and automatically put the unmanned aircraft in advantageous trajectories, flight paths and positions which put unmanned aircraft in the better position to strike due to targeting positions advantages ( McGrew et. al., 2012). Unmanned Aerial Systems are built to function in environment hostile to humans. They are equipped with technologies and features that mitigate human errors and enhance mission capabilities. They provide a solution to human factors elements such as fatigue, cognitive loads, physical and social stressors (Guastello, 2014).The final element that renders unmanned systems superior to manned systems is the safety factor. Unmanned aerial systems present no threat to pilot and crew lives. With the loss of life being a factor that is mitigated by unmanned aircraft systems, they are better suited for air-to-air combat applications.
References
NASA. (2015). Airplane parts and function. Retrieved from https://www.grc.nasa.gov/www/k-
Valavanis K.P., Vachtsevanos G.J. (2015). Handbook of Unmanned Aerial Vehicles. Dordrecht,
Netherlands: Springer Publishing Company. Kratos. (n.d.). XQ-58A Valkyrie. Retrieved
McGrew, J. S., How, J. P., Williams, B., & Roy, N. (2010). Air-combat strategy using
approximate dynamic programming. Journal of Guidance, Control, and Dynamics, 33(5),
1641-1654. doi:10.2514/1.46815
Guastello, S. J. (2014). Human Factors Engineering and Ergonomics: A Systems Approach
(2nd Ed.) Boca Raton, FL: CRC Press of the Taylor & Francis Group. Kelly, M. (2016).
Performance Measurement during Simulated Air-to-Air Combat. Human Factors : The Journal
of the Human Factors and Ergonomics Society., 30(4), 495–506.
Trsek, R. B. (2008, May 3). The last manned fighter: replacing manned fighters with unmanned
combat air vehicles. Wright Papers, (32), https://link-gale-
&xid=018d8bfb
Ashokkumar, C. R. (2019). Unmanned air vehicles for targeting tasks. Proceedings of the
Institution of Mechanical Engineers. Part G, Journal of Aerospace Engineering, 233(5),
1926-1934. doi:10.1177/0954410018755258
Nise, N. S. (2008). Control systems engineering (fifth ed.). Hoboken, NJ: John Wiley & Sons.
Barron, L. G., Carretta, T. R., & Rose, M. R. (2017;2016;). Aptitude and trait predictors of
manned and unmanned aircraft pilot job performance. Military Psychology, 28(2), 65-77.
doi:10.1037/mil0000109
Jaussi, J., & Hoffmann, H. (2018). Manned versus unmanned aircraft accidents, including
causation and rates. International Journal of Aviation, Aeronautics, and Aerospace, 5(4),
3. doi:10.15394/ijaaa.2018.1262
Carretta, T. R. (2013). Predictive validity of pilot selection instruments for remotely piloted
aircraft training outcome. Aviation, Space, and Environmental Medicine, 84, 47–
53. 10.3357/ASEM.3441.2013
Anderson, J.D. (2016). Introduction to Flight. New-York, NY: McGraw-Hill Education
Hibbeler, R.C. (2007). Engineering Mechanics: Dynamics. Upper Saddle River, NJ: Pearson Prentice Hall
Comments